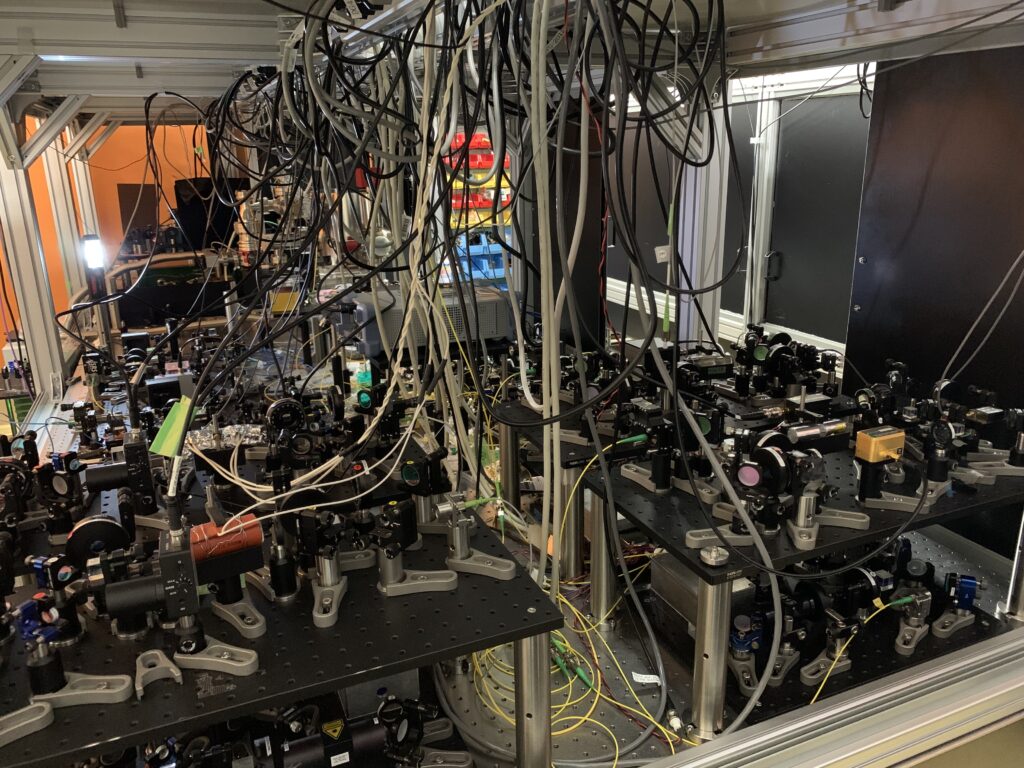
Fundamental Symmetry
Exploring New Physics with Quantum Precise Spectroscopy on Ultracold Heavy Atoms
We aim to unravel the origin of matters in the early universe—including the mechanism behind the disappearance of antimatter and the nature of dark matter—from the perspective of breakings in fundamental symmetries.
In heavy atomic nuclei with extreme structures, relativistic effects and nuclear deformation significantly amplify subtle symmetry violations. These nuclei thus serve as powerful microscopes for probing fundamental symmetries.
By producing such heavy elements via nuclear reactions and applying laser cooling techniques to precisely control their quantum states, we conduct high-precision quantum measurements using atomic interferometers.
- Search for the permanent electric dipole moment (EDM) using optical lattice-based atomic interferometry
- Anapole moment experiments to probe weak interactions inside the nuclear medium
- Development of hybrid-atom comagnetometers for highly sensitive magnetic field measurements
are the ongoing projects.
Francium and Its Production
Among heavy atoms, the main target of our group is Francium (Fr). Fr is the 87th element in the periodic table and is the heaviest alkali element discovered as of April 2025, which encourage the functionality as a microscope.
Unlike more familiar alkali metals such as lithium (Li), sodium (Na), and potassium (K), francium has no stable isotopes. Therefore, it cannot be obtained in sufficient quantities from natural sources and must instead be artificially produced using an accelerator.
At the Fundamental Symmetry Group, we are developing an experimental setup to generate Fr atoms at the RIKEN accelerator facility and trap them in a vacuum using laser light.
Although several methods for producing Fr are known, we use a highly efficient method suitable for small-scale domestic accelerators. In this method, an ion beam of oxygen (O) is directed at a gold (Au) target to induce a nuclear fusion reaction.
Using an accelerator called the AVF cyclotron, the O beam is accelerated to approximately 100 MeV (about 10,000 km/s, roughly 3% the speed of light) and bombarded onto the Au target. As a result, the compound nucleus Fr-215 is formed at a rate of about 0.001%.
The produced Fr atoms diffuse through the solid Au target when it is heated close to its melting point (about 1000 °C), and after around 100 seconds, they reach the surface and eventually desorb as ions. By applying a positive voltage to the Au target, the ions can be extracted as a francium ion beam (Figure 2-1).
To realize this mechanism, we developed a surface ionization ion source (Figure 1-1) in 2019. In the following year's experiment, we achieved a francium ion beam of Fr-210 at a record domestic intensity of 6.7×10⁶ ions per second (Figure 1-3).
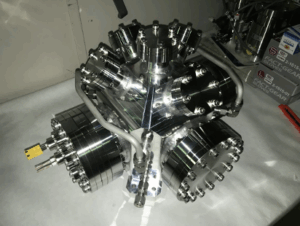
Fgure 1-1. Vacuum chamber of the surface ionization ion source.
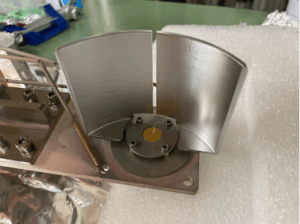
Figure 1-2. Gold target (the golden disc at the center) and the "takefune" electrode (the arc-shaped, folding screen-like structure) used to shape the ion beam.
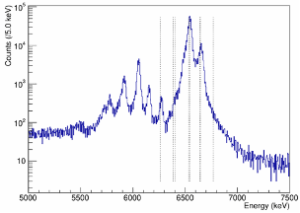
Figure 1-3. Result of counting alpha particles emitted from a metal plate irradiated with the ion beam extracted from the surface ionization ion source, categorized by particle energy. A distinct peak appears near 6500 keV, which is attributed to Fr.
To convert the produced ions into neutral atoms and capture them, we use a neutralizer that facilitates charge exchange with a metal surface, as well as a magneto-optical trap (MOT). These components are assembled and connected to the accelerator beamline to carry out the experiments (Figure 1-4). Unlike nuclear scattering experiments, our experiments deal with extremely low energy scales, such as ion beams with energies around 10 eV and atoms cooled to temperatures of several hundred microkelvin (μK). Moreover, unlike typical cold atom experiments, all apparatus must be operated in a radiation environment. Additionally, since Fr atoms can only be used during beam time, experimental optimization must be carried out using other atomic species. We are addressing these challenges by applying our ideas and continuously refining them through trial and error..
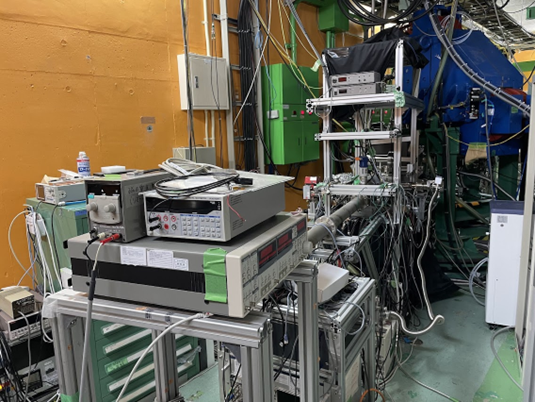
Figure 1-4.
Laser cooling of atoms
One of the laser cooling techniques we employ is Doppler cooling. As illustrated in Figure 2-1, laser light with a frequency slightly lower than the atomic resonance is used.
When an atom absorbs a photon, it is excited and receives the photon's momentum, causing it to decelerate.
After a short time, the atom spontaneously emits a photon and returns to its ground state. Although the atom receives a recoil from this emission, the direction of spontaneous emission is random, and thus the recoil momentum averages out to zero over time.
By repeating this absorption–emission cycle tens of thousands of times, atoms moving at room temperature speeds (~300 m/s) can be slowed to just a few meters per second.
When counter-propagating lasers are applied along three orthogonal axes, atoms can be cooled in all three spatial directions.
Additionally, a magneto-optical trap (MOT) that combines this laser cooling with a magnetic field gradient created by anti-Helmholtz coils allows atoms to be spatially confined at temperatures of several hundred microkelvin (μK).
Figure 2-2 shows a fluorescence image of Rb atoms cooled to around 100 μK, captured with a camera.
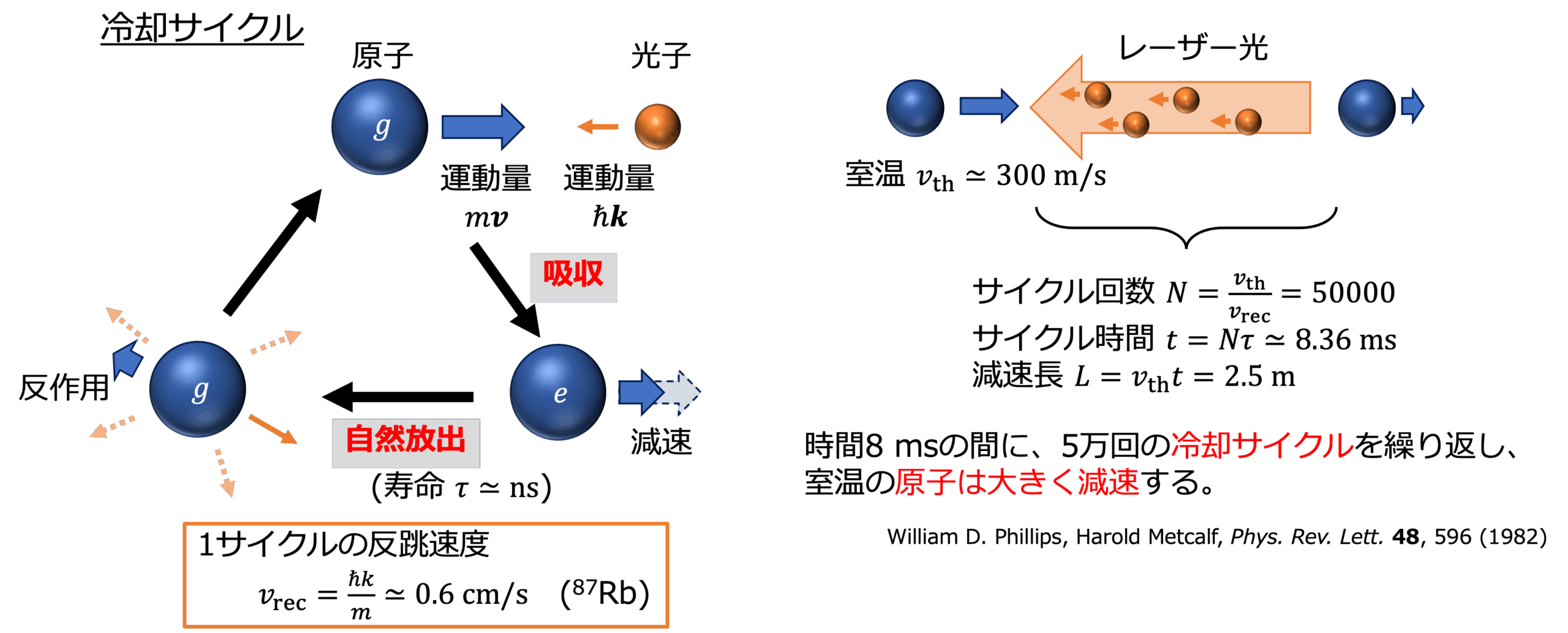
Figure 2-1.
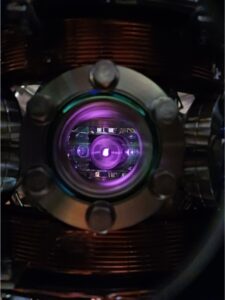
Figure 2-2.
Francium (Fr) has no stable isotopes and, as mentioned above, must be produced via nuclear fusion reactions using an accelerator. As a result, experiments involving Fr atoms can only be conducted during the limited periods when the accelerator is in operation.
To prepare for precision Fr-EDM (Electric Dipole Moment) measurements, we are conducting research and development using stable alkali atoms such as rubidium (Rb) and cesium (Cs) in our laser spectroscopy laboratory.
To perform laser cooling and precision spectroscopy of atoms, it is essential to prepare lasers tuned to the specific energy levels of each atomic species and to stabilize their frequencies. Accordingly, we have developed lasers matched to the transition frequencies of Rb, Cs, and Fr respectiveley, and constructed spectroscopy systems and electronic circuits that employ absorption lines in gas cells for feedback control. These narrow-linewidth lasers are operated on a daily basis (Fig. 2-3).
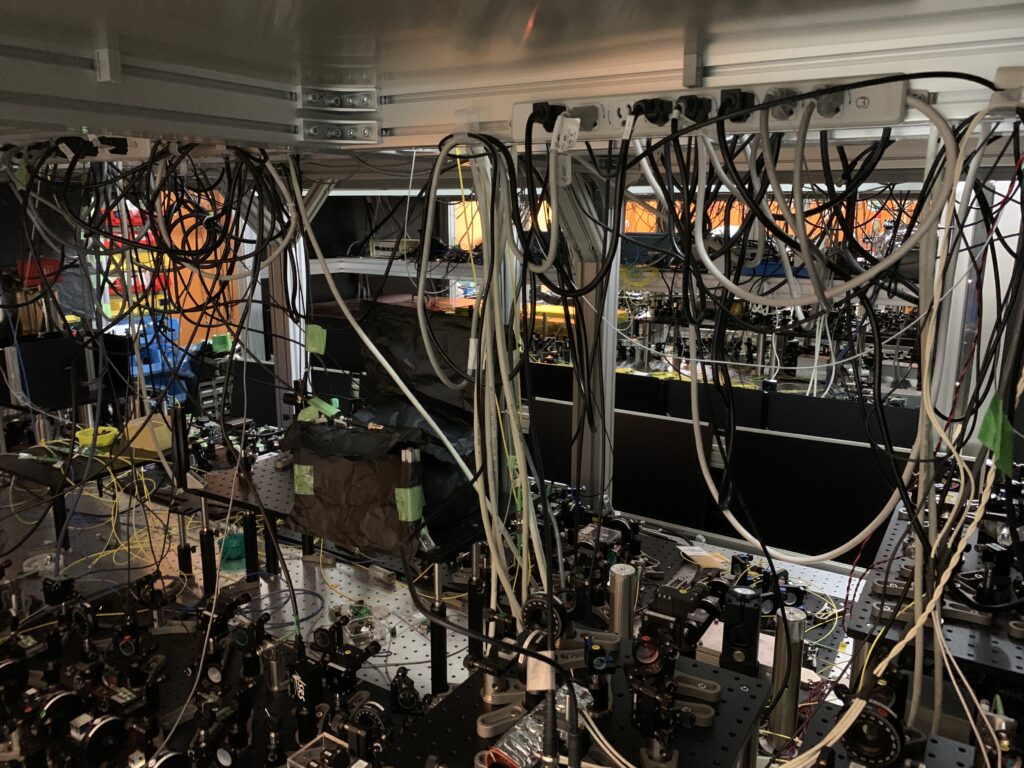
図2-3.
Optical Lattice Co-Magnetometer
For precision atomic spectroscopy, however, the near-resonant lasers used for Doppler cooling can disturb the quantum states of atoms.
To address this, atoms are transferred into an optical lattice—a potential formed by standing waves of far-off-resonant laser light.
The atoms interact with the electric field of the non-resonant laser, causing an energy shift known as the Stark shift.
The stronger the electric field, the lower the atomic energy levels, causing atoms to be trapped in the intensity maxima (antinodes) of the standing wave.
When francium atoms are cooled below the Doppler limit and trapped in such an optical lattice, they become powerful probes for investigating violations of fundamental symmetries in nature.
This is because relativistic effects and nuclear deformation—particularly pronounced in heavy atoms like Fr—can greatly enhance symmetry-violating signals. This enhancement is often likened to a "microscope" for fundamental symmetry violations (Figure 3-1). However, this microscopic sensitivity also makes the system extremely susceptible to external perturbations such as magnetic and optical fields. It is therefore essential to detect and correct for these disturbances. This leads to the concept of the optical lattice co-magnetometer (Figure 3-2). In this approach, lighter atoms, which do not significantly amplify symmetry violations, are also trapped in the same optical lattice.
In 2024, we successfully trapped Rb and Cs simultaneously in the same optical lattice.
Using spin precession frequency measurements based on the Faraday rotation effect—a method known for enabling quantum non-demolition measurements—we were able to simultaneously extract both magnetic and optical fields.
We are currently working to further improve measurement precision and are also exploring novel techniques that utilize unique quantum phenomena.
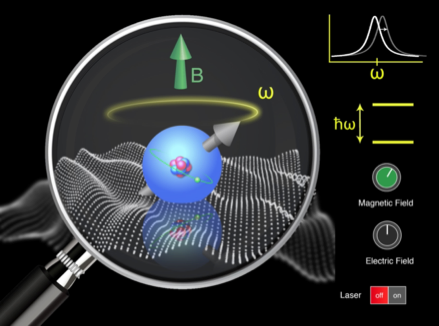
図 3-1.
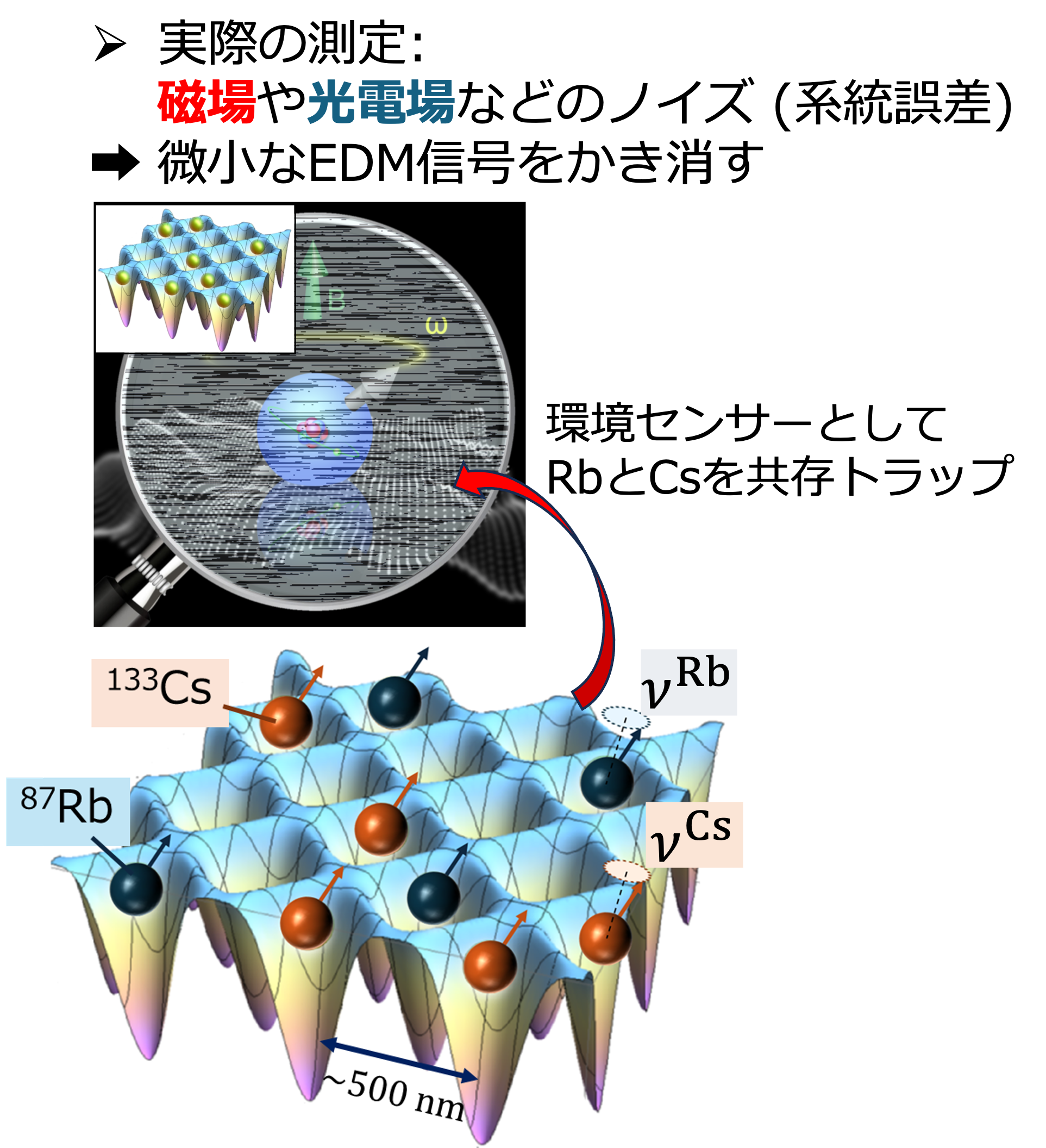
図 3-2.
Laser cooling experiments are a type of tabletop experiment, and due to their relatively small scale, individual ideas and creativity play a major role in driving the research forward. The laser sources, electronic circuits, and vacuum systems used in this study are all designed and developed in-house, making it especially rewarding to conduct experiments using equipment we have built ourselves.
Development of Another Isotope: 221Fr
Fr-221 is an unstable isotope with a half-life of approximately 5 minutes, produced via alpha decay of Ac-225. Because Ac-225 itself has a half-life of about 10 days and continuously emits Fr-221 over an extended period, experiments using Fr-221 can be conducted over several weeks without the need for an accelerator—unlike with Fr-210.
Moreover, unlike Fr-210, Fr-221 has a half-integer nuclear spin, which allows its nucleus to exhibit an electric dipole moment (EDM) originating from quarks.
By performing measurements with both Fr-210 and Fr-221 in parallel, there is potential to probe the electric dipole moment of the quarks.
We are currently developing techniques for the trapping and cooling of Fr-221 in preparation for high-precision spectroscopic measurements (Figure 4-1).
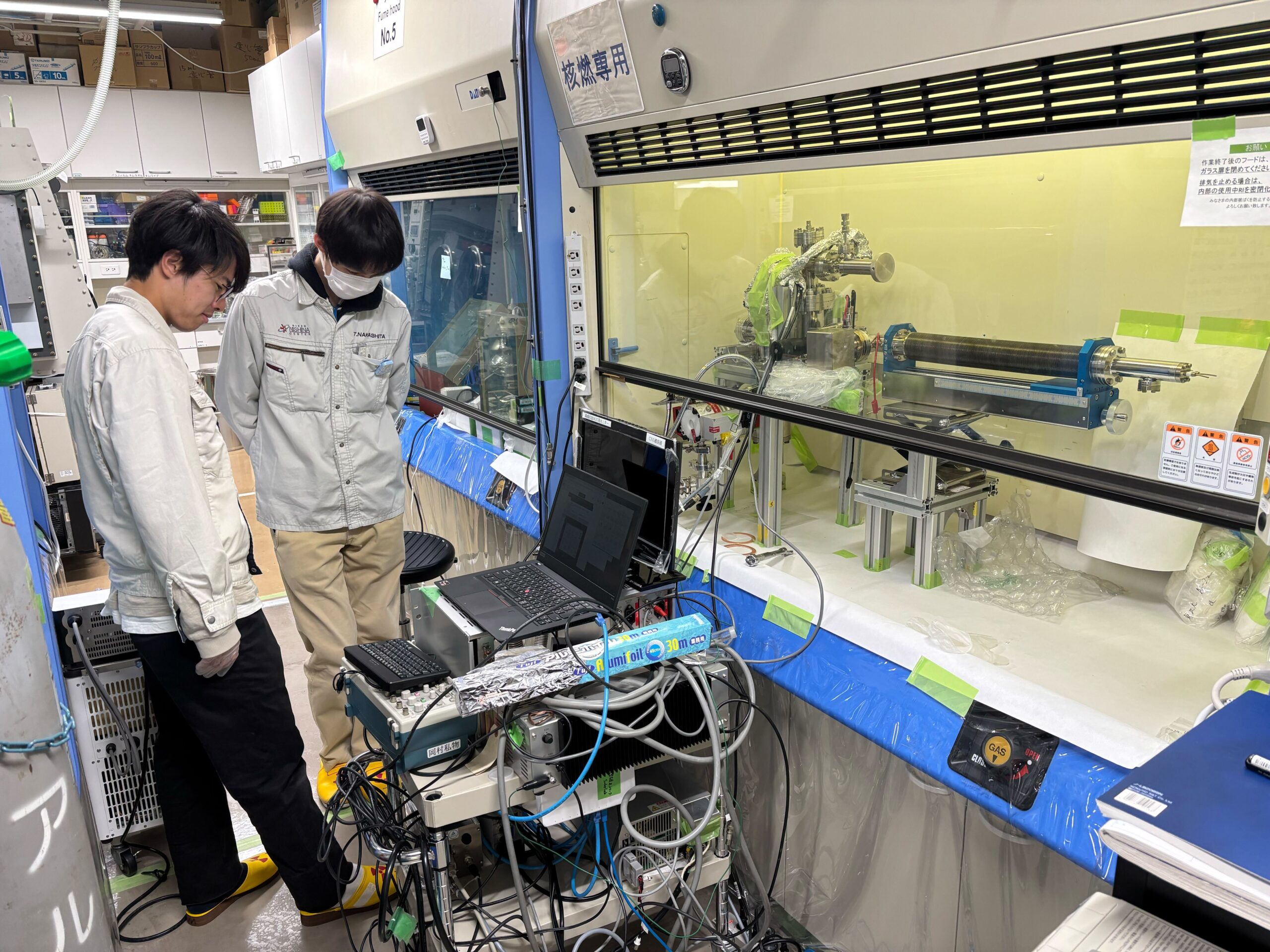
Figure 4-1. Development of Laser cooling of Fr-221